Detection of cosmic dust in the Earth's upper atmosphere
Mark Hervig, GATS Inc., Driggs, Idaho.
It is estimated that nearly 44 metric tons of
meteoric material enter the Earth�s atmosphere daily [Hughes, 1975;
Millman, 1975]. Approximately ~70% of the incident meteoroids are
vaporized due to heating during atmospheric entry at altitudes between
80 and 100 km. Meteorites are the surviving portion of a meteoroid,
and micrometeorites are particles that are too small to reach their boiling
point. Meteoric materials are oxidized by collision with atmospheric
O2, and vapor resulting from meteoroid
ablation condenses to form �smoke� particles in the mesosphere [e.g., Rosinski
and Snow, 1961; Hunten et al., 1980]. The composition
of incident meteoroids is similar to carbonaceous chondrites containing
an abundance of volatile elements including silicon, magnesium,
and iron. While the incident mass of cosmic material is primarily
from meteoroids with radii between 50 and 500 mm
[Millman, 1975], the suspended atmospheric mass exists primarily
as smoke particles with radii between 0.2 and 10 nm [Hunten et al.,
1980]. This work explores the detection of cosmic smoke and dust
at mesospheric altitudes using solar occultation at infrared wavelengths.
1. Optical Constants of Smoke and Dust Analogues
While the exact composition of smoke particles is unknown,
they are thought to contain a variety of compounds including carbon,
sulfur, silicon, magnesium, and iron. Suggested
smoke particle compositions include oxides of the volatile compounds (e.g.,
FeO and SiO2). Optical constants have
been measured for a variety of smoke analogues [e.g., Jager et al.,
1998; Henning and Mutschke, 1997] and the Astrophysical
Institute at the Friedrich-Schiller-Universität, Jena has
compiled an extensive record of optical constants for a variety of particles
composed of silicates, oxides and sulfides, and carbon.
Examples of these data are given in Figure 1.
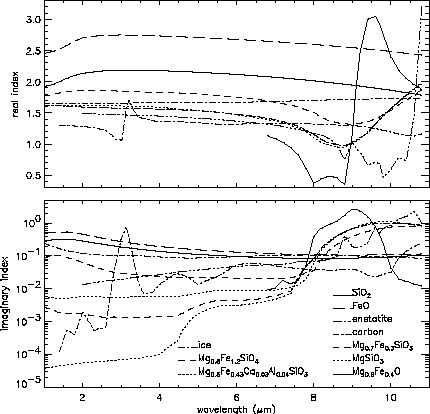 |
Figure 1. The real and imaginary refractive
indices for ice, and a variety of cosmic smoke analogues. |
2. The Distribution of Smoke in Size and Altitude
Complete size distributions of smoke and dust in the
atmosphere have not been measured, nor has the distribution of these
particles in altitude. Thus, the current understanding of these
particles is derived from scant observations combined with theory.
Hunten et al. [1980] offered model calculations of height dependent
smoke and dust size distributions, based on the incident meteoric
mass flux, velocity distribution, ablation, and recombination
of ablation products. Rapp et al. [2001] have recently modeled
the vertical profile of smoke particle size distribution using the Community
Aerosol and Radiation Model for Atmospheres (CARMA). That work used
the influx of meteoric material from Kalashnikova et al. [2000],
and assumed that all incoming material ablates to form particles with initial
radii of 0.25 nm. These particles coagulate to form larger particles,
and their distribution in height is determined by the vertical wind [Koerner
and Sonnemann, 2001] and eddy diffusion [Luebken,
1997]. The volume of smoke versus altitude from the CARMA model is
shown in Figure 2a. The greatest abundance of cosmic particles occurs
near 84 km altitude, and the particle size distribution at this height
is shown in Figure 2b. By comparison, volume densities in typical
polar mesospheric clouds (PMCs) are roughly 0.1 mm3
cm-3, and PMC particles are roughly
100 times larger than smoke, although smoke particles are more numerous
(Figure 2b).
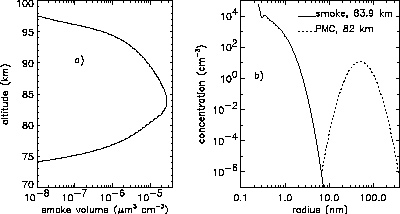 |
Figure 2. a) The volume of cosmic smoke
versus altitude from model calculations. b) The smoke size distribution
at 83.9 km. A typical PMC size distribution is shown for comparison.
The PMC volume density for this distribution was 0.08 mm3
cm-3. |
3. Remote Sensing of the Smoke Layer
Because the smoke layer is tenuous, the best approaches
for remote detection will likely involve scattering and/or absorption of
radiation from a strong source. Two measurement approaches within
this category are lidar and solar occultation. Here we consider smoke
detection using solar occultation at infrared wavelengths. Advantages
for this approach become evident below. The Solar Occultation for
Ice (SOFIE) instrument has been proposed for making infrared measurements
of aerosols and gasses at mesospheric altitudes. The 8 SOFIE wavelengths
(2.3 to 10 mm) were considered in smoke signal
simulations.
Smoke particles will attenuate incident solar radiation
through scattering and absorption (extinction). Smoke extinction
spectra were modeled based on the particle refractive indices and size
distributions discussed above. Assuming spherical particles,
the extinction cross sections of individual particles were calculated using
Mie theory. Extinction coefficients b(l)
were then determined by integrating the single particle cross sections
over an appropriate size distribution. Calculated smoke extinction
spectra considering a model smoke size distribution and a variety of particle
compositions are shown in Figure 3.
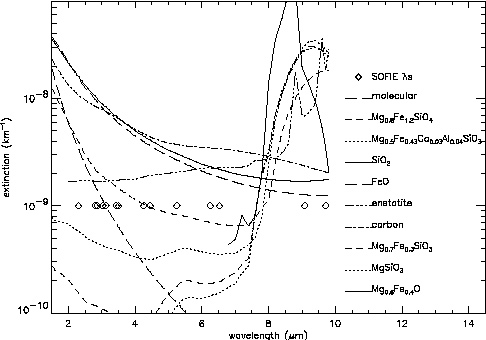 |
Figure 3. Extinction versus wavelength
calculated using the model smoke size distribution at 83.9 km (Figure 2)
and refractive indices for a variety of particle types. Also shown
is the molecular extinction calculated for 160 K temperature and 0.004
mb pressure. SOFIE channel locations are indicated. |
References
Bertie, J. E., H. J. Labbe, and E. Whalley, Absorptivity of ice I in
the Range 4000-30 cm-1, J. Chem. Phys., 50, 4501-4520, 1969.
Biermann, U. M., T. Presper, T. Koop, J. MoBinger, P. J. Crutzen, Th.
Peter, The unsuitability of meteoric and other nuclei for polar stratospheric
cloud freezing, Geophys. Res. Letters, 23, 1693-1696, 1996.
Henning, Th., B. Begemann, H. Mutschke, J. Dorschner, Optical Properties
of Oxide Dust Grains, Astron. Astrophys. Suppl. Ser., 112, 143-149, 1995.
Henning, Th., and H. Mutschke, Low-temperature infrared properties
of cosmic dust analogues, Astron. Astrophys., 327, 743, 1997.
Hunten, M. D., R. P. Turco, O. B. Toon, Smoke and Dust particles of
meteoric origin in the mesosphere and stratosphere, J. Atmos. Sci.,
37, 1342-1357, 1980.
Hervig, M. E., R. E. Thompson, M. McHugh, L. L. Gordley, J. M. Russell
III, and M. E. Summers, First confirmation that water ice is the primary
component of polar mesospheric clouds, Geophys. Res. Letters, 28, 971-974,
2001.
Jäger, C., H. Mutschke, J. Dorschner, and Th. Henning, Optical
properties of carbonaceous dust analogues, Astron. Astrophys., 332, 291,
1998.
Kalashnikova, O., M. Horanyi, M., G. E. Thomas, O. B. Toon, Meteoric
smoke production in the atmosphere, Geophys. Res. Lett., 27, 3293-3296,
2000.
Korner, U., and G. R. Sonnemann, Global three-dimensional modeling
of the water vapor concentration of the mesosphere-mesopause region and
implications with respect to the noctilucent cloud region, J. Geophys.
Res., 106, 9639-9652, 2001.
Lübken, F.-J., Seasonal variation of turbulent energy dissipation
rates at high latitudes as determined by in situ measurements of neutral
density fluctuations, J. Geophys. Res., 102 , p13,441, 1997.
Mutschke, H., B. Begemann, J. Dorschner, C. Jäger, and Th. Henning,
Optical data of glassy pyroxenes and olivines, The Cosmic Dust Connection,
ed. J. M. Greenberg, Kluwer, Dordrecht, p223, 1996.
Rapp M., F.-J. Luebken, A. Muellemann, G. E. Thomas, and E. Jensen,
Small scale temperature variations in the vicinity of NLC: Experimental
and model results, J. Geophys. Res., in review, 2001.
von Cossart, G., J. Fiedler, and U. von Zahn, Size distributions of
NLC particles as determined from 3-color observations of NLC by ground-based
lidar, Geophys. Res. Lett., 26, 1513-1516, 1999.